Thank you for visiting nature.com. You are using a browser version with limited support for CSS. To obtain the best experience, we recommend you use a more up to date browser (or turn off compatibility mode in Internet Explorer). In the meantime, to ensure continued support, we are displaying the site without styles and JavaScript.
npj Clean Water volume 6, Article number: 73 (2023 ) Cite this article Cleaning Liquid
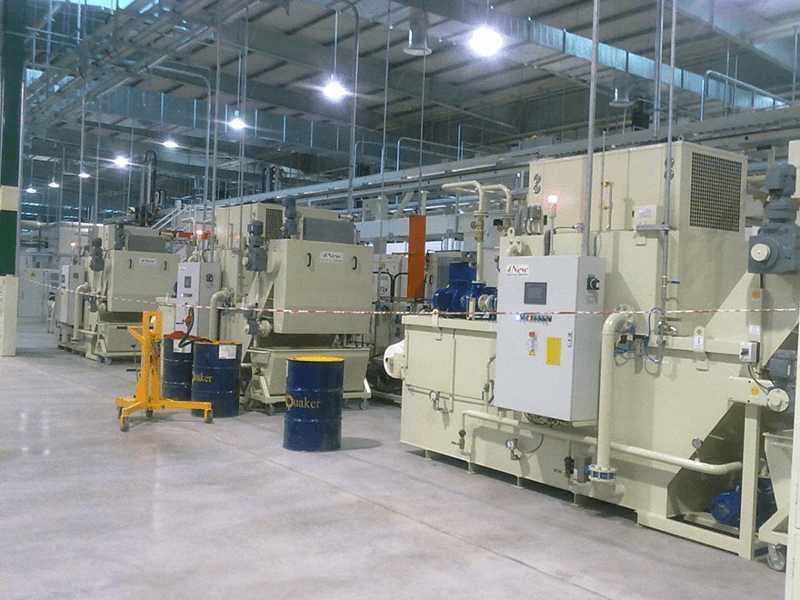
Green walls are gaining attention for greywater management in the imminent terrestrial space and land constraint scenario. They have been tested primarily with greywater from a single source such as showers, hand or wash basins, laundry, and kitchen or a mix of a couple of these sources but barely with mixed greywater from all these household activities. Here, a green wall system coupled with a slow sand filter (SSF) was tested for managing household greywater. It consisted of a set of five serial hydraulic flow-connected reactors and an SSF unit. Each reactor housed an Epipremnum aureum sapling embedded in the support bed matrix, consisting of cocopeat and granular activated charcoal. The system operated at 150 cm d−1 hydraulic loading rate (HLR) achieved 90 ± 0.7%, 85 ± 4.5%, 72.9 ± 4.4%, and 60.6 ± 5.1% removal efficiencies for turbidity, chemical oxygen demand (COD), total nitrogen (TN), and total phosphorous (TP), respectively. The system maintained similar treatment performance with varying greywater strength when COD and TN were below ~400 and ~15 mg L−1, respectively. The polished effluent produced by SSF operated at 187 cm d−1 HLR, with characteristics <5 mg L−1 COD, <2 NTU turbidity, <1 mg L−1 TN, ~0.5 mg L−1 TP, ~7.8 pH, and <100 MPN per 100 ml fecal coliforms, qualifies the standards for non-potable reuse applications. Along with reclaimed water reuse, green walls provide environmental benefits by fixing CO2 in plant biomass. Overall, the low-cost system offers efficient greywater management in an eco-friendly way with minimized resource consumption and areal footprint.
Naef AA Qasem, Ramy H. Mohammed & Dahiru U. Lawal
Maria L. Vera, Walter R. Torres, ... Victoria Flexer
Rui Wang, Kai Yang, … Song Jin
The overexploitation of freshwater reservoirs to cater to the demands of expanding agricultural and industrial activities for sustaining the booming human population has created freshwater scarcity in most countries. According to the UN-Water 2021 report, around 2.3 billion people live in water-stressed nations, of which 733 million live in high and critically water-stressed regions. The pollution of surface water bodies by mixing untreated or partially treated wastewater due to the inadequacy of treatment infrastructure in most developing countries has further intensified the water crisis. These scenarios demand urgent measures to manage water and wastewater resources sustainably. UN-World Water Development Report (2017) highlighted that safely managed wastewater is a sustainable source of water, energy, and nutrients. Decentralized wastewater management approaches that offer water reuse options are considered a promising way forward across the globe to managing crucial water resources, as confirmed by life cycle assessment analyses1. Separating and managing different types of wastewater at the source is acknowledged as a sustainable approach to addressing wastewater management-related challenges2,3. To this end, greywater (GW) recycling has gained attention in the past decade. GW can be easily treated since it generally contains a lower load of contaminants like organics, nutrients, and pathogens than toilet mix wastewater or blackwater4,5,6. Managing source-separated GW lowers the burden on centralized treatment facilities by reducing the wastewater volume and thus minimizing the energy requirement. GW recycling at source for non-potable reuses like toilet washing and landscape gardening, which would otherwise require freshwater, is a plausible strategy to mitigate the water scarcity issue. Reducing dependency on freshwater usage in this way could contribute to achieving water sustainability and resiliency7.
Different treatment approaches involving technological solutions based on physical, chemical, and biological processes and their combinations have been tested for GW treatment8,9,10,11,12,13. Lately, research on ecological principles or nature-based solutions (NBS) for wastewater management is at the forefront due to associated sustainability, esthetics, and microclimate benefits. Nature-based technological solutions work based on the natural cleansing actions of diverse microbes and plants along with the physico-chemical processes that occur in the support bed matrix14. An extensive review by Boano et al. (2020) provides deep insights into the viability of different NBS like constructed wetlands, green roofs, and green/living walls for GW management by covering key aspects such as treatment performance, hydraulic designs, and life cycle assessment and environmental benefits14. Integrating NBS with other emerging sustainable technologies like hydroponics and microbial fuel cells is also considered for wastewater management and energy or food recovery15,16,17,18,19. Among various nature-based technological solutions, green or living walls and roofs are getting attention since these do not have a land footprint20,21 as opposed to the well-researched traditional constructed wetlands technology, which has a high land footprint14,22. The land is an essential asset of our life support system and the economy. By 2050, the world’s population is expected to be between 8.5 and 9.9 billion, with 55‒78% of the population residing in urban areas23,24. The terrestrial space accessibility and availability without affecting natural biodiversity habitats is anticipated to become a matter of concern to meet the needs of the booming population in the upcoming decades. Hence, a holistic approach involving sustainable resource management practices is imperative in all sectors, including wastewater management. Extensive research efforts are thus put into making NBS more sustainable in terms of minimized resource consumption but increased resource recovery, thereby making them economically feasible.
Research on green walls and green roofs has been conducted with GW either from a single source such as showers, hand basins, laundry, and kitchen or a mix of a couple of these sources14,20,21. Different GW sources that have been reported in green walls and green roofs include, for instance, wastewater from hand basins25, shower + lavatory26, bath showers + wash basins + washing machine27, laundry28, bath showers + bathroom29, dormitory30, kitchen31, and bathroom + kitchen32. The performance of green walls with a household GW, i.e., wastewater from all the household GW sources, namely bathroom, hand basins, laundry, and kitchen sink, remains barely studied. The approach of managing household GW seems more plausible in terms of economics and implementation, considering the current infrastructure of domestic dwellings as compared to targeting the individual GW source. To this end, the present study aimed to evaluate the green wall system coupled with a slow sand filter (SSF) (Fig. 1) for household GW (Table 1) treatment. The cost-effective SSF unit treatment was investigated to remove the microbial load and further polishing the effluent. In addition, the effect of varying hydraulic loading rates and GW strength, which are generally observed in real-world scenarios, on the treatment performance of the system was investigated. In order to check the feasibility of its implementation in households and its influence on the surroundings, a preliminary cost analysis and sustainability assessment of the tested GW recycling system was conducted.
The greywater flow is shown in red-colored arrows.
The GW treatment performance of the green wall―SSF system was investigated systematically in three phases, each with specific objectives and experimental conditions (Table 2).
Hydraulic loading rate (HLR), or correlated hydraulic retention time (HRT), is one of the crucial factors affecting a system’s treatment performance. Figure 2 shows the GW treatment performance in terms of conventional pollutants removal achieved by the green wall system at different HLRs. With the increase in HLRs (from 75 to 300 cm d−1), a slight decrement in chemical oxygen demand (COD) removal efficiencies was observed. This observation is consistent with the findings in other studies33,34. Low HLRs prolong the contact time for microbes to degrade organics. The key processes contributing to COD removal include microbial aerobic degradation in the upper zone and anaerobic degradation in the bottom anoxic zone. The bed matrix acts as a suitable substratum for microbes and facilitates the microbial degradation of organics in the reactor units. In addition, the organic matter of the GW used in this study had good biodegradability with a BOD5:COD ratio of ∼ 0.55, most likely due to the mixing of kitchen wastewater (Table 1). Turbidity removal was mostly in the similar range (86‒93%) at different tested HLRs, as the physical filtration by the bed matrix is the key contributing process to it. Total phosphorous (TP) removal efficiency varied between 55 to 60 %, irrespective of tested HLRs. The major process contributing to phosphorus removal is physicochemical adsorption onto the bed matrix along with plant uptake. Total nitrogen (TN) removal did not show a clear trend with the change in HRLs. TN removal efficiency first increased from 75 to 150 cm d−1 HLRs, then decreased at further high HLRs (Fig. 2). TN removal, if the N contamination is in the form of ammonium ions, requires nitrification and denitrification processes to happen. However, the influent GW used in this study had organic-N (~80% of TN) as a major fraction of N contamination (Table 1). Hence, its removal requires an additional microbial ammonification process, i.e., conversion of organic-N to inorganic-N such as NH4+. Though the increase in HLR can facilitate the ammonification process by passively aerating the upper zone due to the increased flow, it can hamper the denitrification process by reducing residence time in anoxic conditions. Hence, there should be a dynamic balance in operating conditions for these microbial processes to achieve maximum N removal35. Along with microbial N removal, direct uptake of ionic N forms by the plant is a key contributing process to nitrogen removal.
Pollutant removal efficiencies are presented as mean values ± standard deviations of multiple data points (at least four from each system) from two similar performing systems. COD chemical oxygen demand, TP total phosphorous, TN total nitrogen.
The removal of COD, turbidity, TN, and TP differed considerably at different HLRs. The GW treatment performance at the 150 cm d−1 HLR was either at par or higher than the other tested HLRs, considering the different monitored parameters, and thus, it was selected for the long-term system performance evaluation experiment in phase II. The removal efficiencies achieved by a set of planted reactor units for COD, turbidity, TN, and TP at 150 cm d−1 HLR were 85 ± 4.5%, 90 ± 0.7%, 72.9 ± 4.4%, and 60.6 ± 5.1%, respectively. This treatment performance is comparable to or better than reported studies conducted with real greywaters. For instance, Svete (2012) reported 34% and 77% removal of TN and TP from the dormitory GW with lettuce and marigold as plant components in green wall systems30. In another study, a constructed wetland-based integrated on-site treatment system achieved 70% removal efficiencies for COD and Total Kjeldhal Nitrogen (TKN) from GW sourced from a hostel33. Moreover, the wall cascade constructed wetland system achieved 86% and 57% for COD and TKN removal efficiencies from kitchen wastewater31. In a separate study, the green wall system referred to as Total Value Wall showed removal efficiencies of 90% and 82% for TSS and COD, respectively, from the GW mix of bathroom and kitchen32.
The contribution of each reactor component, i.e., support bed matrix and plants, in the pollutants removal or treatment process was also evaluated. The control condition, i.e., only bed matrix containing reactor units achieved similar removal efficiencies for COD (79.4 ± 3.5%) and turbidity (88.4 ± 1.4%) while considerably lower for TN (51.7 ± 3.9%) and TP (52.6 ± 3%) compared to the planted reactor set at the same HLR of 150 cm d−1 (Fig. 3). From these results, it is clear that the bed matrix primarily comprised of cocopeat and associated microbes is the sole contributor to organics and turbidity removal while a major contributor along with plants in nutrients removal. Cocopeat has been reported to outperform different tested materials such as perlite, Leca balls, sand, date seeds, and spent coffee grounds for organic removal34,36. It traps complex organic matter and facilitates organic degradation and mineralization of complex nutrients by diverse microbial processes in different microenvironments of the reactor unit. The high ion exchange capacity of the cocopeat bed matrix also favors plant nutrient uptake by increasing the retention time of ions37. In this study, Epipremnum aureum contributed around 20% to N removal and 10% to P removal at a much lesser retention time of 1.6 h. Wolcott et al. (2016) reported similar TN (25%) and TP (15%) removal efficiencies within 24 h from brewery wastewater having similar average influent TN (7.8–66 mg L−1) and TP (3–4.6 mg L−1) concentrations using E. aureum planted green wall system38. Plant nutrient uptake is a dynamic process and depends on several factors such as concentration of nutrients, availability of the readily utilizable forms of nutrients, physiochemical characteristics of nutrient solution, and environmental conditions39.
Pollutant removal efficiencies are presented as mean values ± standard deviations of multiple data points (at least four from each system) from two similar performing systems. COD chemical oxygen demand, TN total nitrogen, TP total phosphorous.
The performance robustness to the varying GW strength, a scenario generally seen in the real world, is a crucial aspect to determine the practical applicability of the system. Figure 4 shows the GW treatment performance of the green wall system coupled with SSF operated for 15 weeks at 150 cm d−1 HLR but with varying GW strength. The COD removal efficiency of the green wall system fluctuated around 81.3 ± 5.8% (except on the 3rd and 10th week) when the COD was below 400 mg L−1. A sharp decline (up to 61%) was observed when the COD of GW was more than 400 mg L−1 during the 3rd and 10th weeks, as shown in Fig. 4a. The TN removal showed a similar trend to COD removal. The TN removal efficiency fluctuated around 53.5 ± 6.4% when the inlet TN concentration was below 14 mg L−1, while a sharp decline (up to 40%) was recorded when the inlet TN concentration was >14 mg L−1 on the 3rd, 10th, and 12th weeks (Fig. 4b). The pollutant treatment via biological or microbial processes is dependent majorly on the retention time. The retention time of ~1.6 h, with 150 cm d−1 HLR, might not be sufficient to achieve efficient degradation of pollutants at the high loading on some days (i.e., 3rd, 10th, and 12th weeks when inlet GW had more than 400 mg L−1 COD and 15 mg L−1 TN) resulting in decreased COD, TN, and TP removal performance of the green wall system (Fig. 4). The varying GW strength had no considerable effect on turbidity removal efficiency as it remained around 90.2 ± 2.1% (Fig. 4c). It is because turbidity removal involves a physical filtration mechanism subjected to the porosity of the bed matrix. Furthermore, no clear trend was observed between the TP removal and the varying GW strengths (Fig. 4d), as TP removal efficiencies varied between 29% and 58%, irrespective of the GW strength. It might be due to the dynamic adsorption and desorption behavior of the ionic phosphates, resulting from the mineralization of organic phosphorous, onto the bed matrix during continuous feeding mode.
a COD, b TN, c turbidity, and d TP. Pollutant removal efficiencies are presented as a mean of data obtained from two similar performing systems. COD chemical oxygen demand, TP total phosphorous, TN total nitrogen, SSF slow sand filter, NTU nephlaometric turbidity unit.
In addition to the treatment achieved by the green wall system, the SSF unit contributed to further effluent polishing. In the effluent of SSF, the removal efficiencies for COD, turbidity, TN, and TP reached 89.4 ± 4.2%, 94 ± 1.5%, 61.5 ± 7.3%, and 50.1 ± 7.6%, on all but the high pollutant load days (i.e., 3rd, 10th, and 12th weeks). In a sand filter, straining and sedimentation within the filter media are predominant processes involved in removing fine particle contaminants, thus contributing to further turbidity removal. The removal of dissolved organics can be attributed to adsorption and microbial degradation processes. Some of the remaining nitrates and/or nitrites (5.1‒6.4 mg L−1) in the effluent of reactor units might have been removed through the denitrification process in the anaerobic environment of the SSF and thus accounted for TN removal40. Ionic phosphate removal in SSF can be attributed to precipitation or adsorption onto the iron and aluminum oxides that are generally present in the sand41. Starting with filtration, the combination of physicochemical and diverse microbial processes on the upper surface of sand are the key mechanisms responsible for the removal of contaminants in the SSF42. Overall, the green walls system coupled with SSF could sustain a higher load but up to a limit, i.e., inlet with <400 mg L−1 of COD and 15 mg L−1 of TN. Moreover, it showed good resilience in treatment performance even at a high pollutant load, signifying its robustness to the varying GW strength.
The treatment performance of the SSF unit, aimed at the final polishing of the effluent, is the crucial step in deciding the quality of the final effluent based on which the reuse of reclaimed water can be assigned. The treatment performance of a physical filter generally depends on HLR. In this study, the removal of conventional pollutants by SSF unit was highest at 187 cm d−1 HLR, followed by 374, 562, and 749 cm d−1 (Fig. 5). At 187 cm d−1 HLR, the COD, turbidity, TN, and TP removal efficiencies were 95.1 ± 5.7%, 65.1 ± 3.3%, 71 ± 6.6% and 30.3 ± 5.8%, respectively. Under this condition, the concentrations of COD, turbidity, TN, and TP in the final effluent were <5 mg L−1, 1.9 ± 0.4 NTU, 0.8 ± 0.2 mg L−1, and 0.65 ± 0.2 mg L−1, respectively. With the increase in the HLR, the treatment performance of the SSF decreased. For instance, at 749 cm d−1 HLR, the removal efficiencies and effluent concentration of COD, turbidity, TN, and TP were 53 ± 6.4%, 38.7 ± 4.5%, 40.6 ± 7.5%, and 15 ± 5.2% and 19.7 ± 4.5 mg L−1, 3.4 ± 0.8 NTU, 1.7 ± 0.4 mg L−1, and 0.8 ± 0.2 mg L−1, respectively. On a similar ground, the removal of COD, turbidity, TN, and TP by SSF showed an inverse relation with HLR (Fig. 5). With the increase in HLRs and correspondingly less retention time, substantial decrement in removal efficiencies of COD, TN, and TP was recorded, which is in agreement with the findings of other studies34,43,44.
Pollutant removal efficiencies are presented as mean values ± standard deviations of multiple data points (at least four from each system) from two similar performing systems. COD chemical oxygen demand, TP total phosphorous, TN total nitrogen.
Notably, no considerable difference was recorded in the DO, pH, and fecal coliforms profiles, which varied in the range 6.5‒6.9 mg L−1, 7.7‒7.9, and 0.8‒4.6 × 102 MPN per 100 ml, respectively, in the final effluent produced by SSF at different tested HLRs (Supplementary Table 1). The improved DO in the effluent compared to the influent can be justified by the passive aeration during water percolation through the green wall and SSF units. Approximately 2-log fold removal of coliforms compared to the influent was achieved. SSF is a cost-effective means of removing microbiological contaminants from water. The Schmutzdecke layer, which generally contains predatory bacteria, formed at the top layer of sand has been reported to contribute to removing microbes45. Its formation was also observed as the top layer in the SSF unit in this study (Supplementary Fig. 1).
All the materials used in fabricating the complete system comprising green walls and SSF units tested in this study are mentioned in Supplementary Table 2. Most of the infrastructure was made of lightweight but durable and recyclable materials, i.e., cPVC and PVC. Moreover, the green wall system comprised cost-effective and eco-friendly bed matrix materials like cocopeat and granular activated charcoal. The cost incurred to fabricate the duplicate systems (58 L d−1 treatment capacity) tested in this study was reasonable, i.e., 3550 INR or 44 USD (Supplementary Table 2). Using lightweight cocopeat media aided in their reduced structural support requirements and cost. The electricity consumption was also negligible (~0.003 kWh daily), only needed for a few minutes (~5 min) daily to pump 57.6 L of clarified GW to the reservoir. The estimated capital and recurring cost (yearly) of the 400 L d−1 treatment capacity system totals around 13,600 INR (or 167 USD) and 1000 INR (or 12.3 USD), respectively (Supplementary Table 1). Although comparative economic assessment is not straightforward due to associated uncertainties like varying costs of the same material or resource in different countries or continents, some inferences about the cost-effectiveness of the here-demonstrated system can be made if done on the same ground. For instance, the Hydraloop GW recycling unit costs 4000 USD for a family (Hydraloop, The Netherlands). In the Indian context, the estimated cost for constructing a 1 kLD decentralized wastewater treatment system (without disinfection), based on the aerobic or anaerobic process, is within the range of 340–400 USD, as per the report of the Centre of Science and Excellence, India (www.cseindia.org). The estimated capital cost of the tested system is at par if compared at the same ground, with minimal (~0.080 kWh to pump 400 L GW; Supplementary Note 1) operational costs, which is generally not the case for other conventional technological solutions.
In addition to reduced electricity consumption, the system had no land footprint; however, it requires space on the wall for mounting. The projected wall surface area of a tested GW recycling system (ca. 28.8 L d−1 capacity) was also minimal, i.e., ~0.16 m2 due to compact design (Supplementary Fig. 2). The estimated wall surface area for the 400 L d−1 treatment capacity system is around 2.2 m2 with dimensions of 2.1 m × 1.1 m. This areal footprint is either at par or lower than reported green wall systems for household GW treatment26,28,29,30. This design uses minimal space on bare walls or backyards, allowing easy implementation even in the space-constraint scenarios of both rural and urban settings. HRT directly influences reactor size or material consumption, hence the system cost. The faster rate of treatment, i.e., within less than 2 h, provides the tested system with an additional economic advantage. Overall, the demonstrated system in the present study is cost-effective as its fabrication and operation incur minimal and low-cost resources.
With minimized resource consumption, the complete green walls-SSF system treats GW and recovers a crucial water resource. It produces reclaimed water of reusable quality. The characteristics of effluent produced by the SSF unit at the lower (187 cm d−1) and higher (749 cm d−1) tested HLRs were: <5 and 20 mg L−1 COD, <2 NTU and 3.5 NTU turbidity, <1 and 2 mg L−1 TN, ~0.5 and 0.8 mg L−1 TP, ~7.8 and 7.8 pH, ~6.6 and 6.9 mg L−1 DO, and <100 and 500 MPN per 100 ml fecal coliforms, respectively. There are no clear international and national (except very few like Jordan) guidelines for GW reuse; however, most countries follow the wastewater reuse guidelines. The reclaimed water quality was compared with the average reuse standards mentioned in the study by Reynaert et al. (2020)11 (Supplementary Table 3). The effluent quality produced at higher HLR was found to be in agreement with the criteria or standards set for landscape gardening, while the effluent of lower HLR qualifies for even more stringent reuse standards (except for TP) of toilet flushing, car washing, and floor washing. In addition, the effluent quality meets the updated set of proposed guidelines, formulated by considering the already existing guidelines of WHO, US EPA, and many countries for non-potable reuse of GW for several restricted and unrestricted reuse applications46. Reusing reclaimed water in the afore-mentioned applications can reduce freshwater consumption by up to 50% in a household, thus saving on the water bill and lessening the over-exploitation of freshwater reservoirs. The remaining treated water can be used for groundwater or surface water recharging. This approach of circular water use cycle at the point source reduces the burden on freshwater usage and promotes sustainability practices in water resource utilization.
Besides GW recycling, the green walls-SSF system offers ecological and environmental benefits. Due to the robust nature of E. aureum, it showed substantial growth even in low nutrients-containing GW in the system (Fig. 6). Moreover, the creeper nature of E. aureum allowed the creation of green walls, providing positive effects on the well-being of people and also the cooling effect on the environment. It also produces oxygen at night, unlike other plants that release CO2 during nighttime. Apart from its high esthetic value, it contributes positively to mitigating the effect of greenhouse gas CO2, resulting from organic degradation, by fixing it into green plant biomass. In this study, the calculated reduction in the release of CO2 in the environment was around 6% (Supplementary Note 2). Though the value is low, it will be an impactful intervention if such eco-friendly approaches are implemented widely for GW management.
The monitoring was started after the saplings were transplanted in the green wall reactors.
Though cocopeat is an organic material, its degradation is slow (i.e., in years). The worn-out or shabby cocopeat with trapped nutrients can be used as a soil amendment, a well-reported application, for plant growth. On the other side, the saturated granular activated charcoal can be regenerated at houses via a mix of thermal and mechanical processes (i.e., by shaking media in hot freshwater), which can result in the desorbing of some ions. However, the regeneration rate is slow, which makes it an inefficient process. The other approaches, such as chemical and electrochemical, are fast and efficient but are chemical or cost-intensive and challenging to implement in households47. Hence, one can also opt for using saturated nutrient-rich granular activated charcoal as a soil amendment. Overall, the practice of using exhausted organic bed materials for gardening purposes could be an efficient and ecofriendly way to manage the wastes generated by the system.
This study successfully demonstrated the green wall system design with multiple modular reactor units for GW management of households. Mounting small and lightweight reactor units against the wall is reasonably practical since it will disperse the inclining pressure over the wall in contrast to a high pinpoint inclining pressure exerted when one big reactor unit is mounted. Its modular design allows increasing the number or set of reactor units according to the GW quantity that needs to be treated. Furthermore, it offers adjustability to the space availability and easy troubleshooting and maintenance without compromising the treatment process. Hence, it can be easily scaled and implemented as per the end user’s requirement.
In summary, the nature-based green wall―SSF system demonstrated in the present study is efficient for household GW management in an eco-friendly and sustainable way. The HLR is crucial in determining the treatment performance of the system, i.e., for both the main reactor and SSF units. The system achieved higher pollutant removal efficiencies for conventional water quality parameters at a lower hydraulic loading rate, signifying the role of retention time in the treatment process. Clogging was not noticed in the reactor units during the long-term study of over eight months. It indicates that diverse microbial and plant nutrient uptake processes play an important role in treating pollutants. The modular design can allow easy replacement of the first reactor unit, which is prone to clogging in the long run. The resiliency and performance robustness to the varying GW strength shown by the system suggests its practical applicability. The treated effluent meets stringent worldwide reuse standards for several non-potable applications. E. aureum showed substantial growth even in low nutrient-containing GW, which validates its robust nature. Moreover, its creeper growth feature adds ecological and environmental benefits to the system. Overall, based on successful demonstration and estimated propositions, it can be inferred that the green walls―SSF system could be an economically feasible and easy-to-implement sustainable technological solution for household GW management, even in the imminent terrestrial space or land constraint scenario.
The raw GW was collected daily from individual sources, namely the bathroom, washing machine or laundry, kitchen sink, and hand basin of a house due to the lack of a volunteer test house with a separate GW collection system in our vicinity or nearby region. Though the GW composition depends on lifestyle and water abundance, the collected wastewater was mixed in specific proportions (i.e., laundry 26–27%, bathroom 47–49%, and kitchen with hand basin 24–37%) that different household activities contribute to the total greywater as reported in literature48,49. It was freshly prepared daily and fed to the systems. Before loading to the reservoir, it was passed through a primary treatment procedure consisting of 6 h sedimentation followed by oily scum removal from the top. The physicochemical characterization of the clarified GW used in this study is presented in Table 1 and is as per the reported range in different studies8,10,14.
Two identical green walls coupled with SSF systems were fabricated on plywood and mounted on the wall through wiring/drilling. The individual system had three main components: a reservoir (45 L capacity), green walls consisting of a set of five reactor units, and an SSF unit (Fig. 1). A submersible water pump (40 W) was used to pump the clarified GW to the reservoir, and after that GW flowed to the reactor units by gravity. The set of five reactor units constituted the main pollutant treatment site. Each reactor unit was made of cylindrical PVC pipe with an inner diameter of 7 cm and a length of 20 cm. These dimensions of reactor units were selected based on our prior understanding of size sufficiency for accommodating plant root growth of around one year while managing low nutrient GW from previous studies50,51. The reactor units were connected in a serial hydraulic flow mode, and the water saturation zone was maintained in each reactor. Briefly, the entering GW first percolated through the support bed, then rose along a tube till the water level, and finally drained to the lower unit, and so on, as shown in the schematic (Fig. 1). Water level in the reactor unit was maintained up to 18 cm or just below the bed matrix surface. The main treatment unit of a system constituted around 2 L of total working volume, with each reactor unit having ~0.4 L void or working volume that resulted after filling of bed matrix and plant sapling. For setting up the SSF unit, locally available sand was sieved for two different fractions: fine sand (0.15–0.2 mm size) and coarse sand (1–1.5 mm size). The cylindrical PVC pipe (Ø 7 cm) was filled, from top to bottom, with fine sand for 17 cm height, followed by coarse sand for 3 cm height. The coarse sand was used to restrict the leaching of fine sand from the bottom water drain holes of the SSF unit.
Each reactor unit (except the first one, which was operated as a primary clarifier in non-planted condition) consisted of two major components: support bed matrix and plant sapling. E. aureum (aka Money plant, Golden Pothos, or Devil’s Ivy) was used as a plant component due to its several favoring characteristics. These include adaptability to varying nutrient and light conditions, sufficient perennial growth, creeper growth style, fibrous root structure, and easy propagation52. Apart from its esthetic values, it has been reported to detoxify different contaminated environmental media, i.e., air, soil, and water, through diverse phytoremediation processes52. The previous research also suggested it as a promising plant candidate for various nature-based wastewater treatment technologies as it can grow even in low nutrient conditions like GW53. For the main experiments, similar-sized young rooted saplings with a wet weight of 6.9 ± 1.3 g were transplanted in the reactor units.
For the support bed matrix, tap water washed and dried cocopeat (Source: TrustBasket, India; 2-4 mm particle size; porosity: ~0.47) and granular activated charcoal (Source: Andel Marketing & Services, India; 3–4 mm particle size; porosity: ~0.61) were used. The reactor units were filled with granular activated charcoal and cocopeat in 20:80 proportions (by the length of the reactor unit) from bottom to top. This combination was selected since it outperformed other tested combination proportions of granular activated charcoal, quartz/silica sand, and cocopeat in removing conventional pollutants from domestic wastewater50. Cocopeat is an eco-friendly and cost-effective light-weighted support media with several other characteristics, such as porous nature favoring rhizospheric microbes growth besides high water holding and cation exchange capacity, which aids in pollutant removal54. A small amount (~120 g per reactor unit) of granular activated charcoal, reported to be a promising adsorbent with a high surface area, was used at the bottom of the reactor unit to remove the dissolved conventional and emerging contaminants55.
The experimental period of the whole study is presented in three phases (Table 2). In phase I, the aim was to optimize the HLR to the green walls; hence, the clarified GW characteristics were limited to minimum variation to nullify the influence of GW strength. The phase II experiments were conducted for 15 weeks with varying concentrations of conventional pollutants in clarified GW to check the performance robustness of the system during varying GW strength, which is generally seen in real-world scenarios. In phase III, the effect of HLRs on the effluent polishing performance of SSF was evaluated using the effluent of the green walls as an inlet to SSF. The SSF unit was operated in a water-unsaturated condition. The system was mounted on the wall, and all the experiments were conducted outdoors at the IISER Mohali campus (India) to mimic real-world household settings. In each tested HLR condition in the green walls or SSF unit, one-week acclimation was ensured for physiological adaptions of biota, i.e., diverse microbes and plant sapling, to varying conditions before sampling and analysis work. No specialized microbial inoculum was externally added to the reactor units. The inherent microbes of GW flourished in different microenvironments of the bed matrix. The GW treatment performance in each experimental condition was assessed by monitoring the conventional parameters, viz., COD, TN, and TP, and turbidity on multiple days.
The influent and effluent samples of different tested experimental conditions were analyzed within 12 h of their collection. TN and TP concentrations were estimated by the alkaline persulfate digestion method, followed by absorbance reading using a UV–Vis spectrophotometer (WTW Photolab 7600 UV–Vis, Xylem). Turbidity, pH, and dissolved oxygen (DO) were measured using a digital multiparameter meter (Model: HI9829, Hanna Instruments). COD was analyzed by the closed reflux method. Fecal coliform count was estimated by performing an MPN test adapted from “Standard Methods for the Examination of Water and Wastewater.”
The GW treatment performance is presented as removal efficiencies (R%), calculated according to Eq. (1).
where C0 represents the initial concentration of the specific constituent in the influent (mg L−1), and Cs denotes the concentration of the same constituent (mg L−1) in the effluent.
The systems performed at a similar level; thus, the data are presented as the mean of two replicate systems along with standard deviations.
A basic cost and sustainability analysis was done for the complete system considering different aspects, namely system fabrication cost, electricity consumption, and footprint of different resources such as water, land, and carbon, which are relevant to assessing the system’s sustainability. Based on the findings and understanding developed from the demonstrated system, the estimations on the parameters mentioned above for 400 L d−1 (average amount of GW produced by a family of 4–5 members daily) treatment capacity system were done to assess its sustainability and feasibility for field-scale implementation. For the cost estimation, the current market cost of different resources used in the fabrication of the system was considered (Supplementary Table 2). For estimating electricity consumption, the time required to pump the clarified GW to the reservoir placed at a height (2 m) was considered (Supplementary Note 1). It should be noted that the fabrication cost and electricity consumption of the demonstrated system mentioned in this study was a combined estimation of duplicate tested systems since one reservoir was used in the study. For the carbon or CO2 footprint estimation, the decreased CO2 amount released in the environment due to fixation in plant biomass during the study period was considered (Supplementary Note 2). The total CO2 produced from the organic matter degradation was considered in these calculations.
All the data generated and estimated in this study are presented in the main text and supplementary information file. The primary data are available with the corresponding author upon reasonable request.
Opher, T. & Friedler, E. Comparative LCA of decentralized wastewater treatment alternatives for non-potable urban reuse. J. Environ. Manag. 182, 464–476 (2016).
Larsen, T. A., Udert, K. M. & Lienert J. Source Separation and Decentralization for Wastewater Management (IWA Publishing, 2013).
Moges, M. E., Todt, D., Eregno, F. E. & Heistad, A. Performance study of biofilter system for on-site greywater treatment at cottages and small households. Ecol. Eng. 105, 118–124 (2017).
Friedler, E., Kovalio, R. & Galil, N. I. On-site greywater treatment and reuse in multi-storey buildings. Water Sci. Technol. 51, 187–194 (2005).
Jefferson, B., Palmer, A., Jeffrey, P., Stuetz, R. & Judd, S. Grey water characterisation and its impact on the selection and operation of technologies for urban reuse. Water Sci. Technol. 50, 157–164 (2004).
Nolde, E. Greywater reuse systems for toilet flushing in multi-storey buildings—over ten years experience in Berlin. Urban Water 1, 275–284 (2000).
Yoonus, H. & Al-Ghamdi, S. G. Environmental performance of building integrated grey water reuse systems based on Life-Cycle Assessment: a systematic and bibliographic analysis. Sci. Total Environ. 712, 136535 (2020).
Ghaitidak, D. M. & Yadav, K. D. Characteristics and treatment of greywater—a review. Environ. Sci. Pollut. Res. 20, 2795–2809 (2013).
Ghunmi, L. A., Zeeman, G., Fayyad, M. & van Lier, J. B. Grey Water treatment systems: a review. Crit. Rev. Environ. Sci. Technol. 41, 657–698 (2011).
Oteng-Peprah, M., Acheampong, M. A. & deVries, N. K. Greywater characteristics, treatment systems, reuse strategies and user perception—a review. Water Air Soil Pollut. 229, 255 (2018).
Reynaert, E. et al. Practical implementation of true on-site water recycling systems for hand washing and toilet flushing. Water Res. X 7, 100051 (2020).
Wu, B. Membrane-based technology in greywater reclamation: a review. Sci. Total Environ. 656, 184–200 (2019).
Wurochekke, A. A. et al. Household greywater treatment methods using natural materials and their hybrid system. J. Water Health 14, 914–928 (2016).
Boano, F. et al. A review of nature-based solutions for greywater treatment: applications, hydraulic design, and environmental benefits. Sci. Total Environ. 711, 134731 (2020).
Capodaglio, A. G., Bolognesi, S. & Cecconet, D. Sustainable, decentralized sanitation and reuse with hybrid nature-based systems. Water 13, 1583 (2021).
Rapisarda, R., Nocera, F., Costanzo, V., Sciuto, G. & Caponetto, R. Hydroponic green roof systems as an alternative to traditional pond and green roofs: a literature review. Energies 15, 2190 (2022).
Xu, L. et al. A hydroponic green roof system for rainwater collection and greywater treatment. J. Clean. Prod. 261, 121132 (2020).
Yadav, R. K., Das, S. & Patil, S. A. Are integrated bioelectrochemical technologies feasible for wastewater management? Trends Biotechnol. 41, 484–496 (2023).
Yadav, R. K., Chiranjeevi, P., Sukrampal & Patil, S. A. Integrated drip hydroponics–microbial fuel cell system for wastewater treatment and resource recovery. Bioresour. Technol. Rep. 9, 100392 (2020).
Addo-Bankas, O. et al. Green walls: a form of constructed wetland in green buildings. Ecol. Eng. 169, 106321 (2021).
Pradhan, S., Al-Ghamdi, S. G. & Mackey, H. R. Greywater recycling in buildings using living walls and green roofs: a review of the applicability and challenges. Sci. Total Environ. 652, 330–344 (2019).
Srivastava, P., Yadav, A. K., Garaniya, V. & Abbassi, R. Constructed wetland coupled microbial fuel cell technology. In Microbial Electrochemical Technology 1021–1036 (Elsevier, 2019).
Jiang, L. & O’Neill, B. C. Global urbanization projections for the Shared Socioeconomic Pathways. Glob. Environ. Change 42, 193–199 (2017).
Kc, S. & Lutz, W. The human core of the shared socioeconomic pathways: population scenarios by age, sex and level of education for all countries to 2100. Glob. Environ. Change 42, 181–192 (2017).
Masi, F. et al. Green walls for greywater treatment and recycling in dense urban areas: a case-study in Pune. J. Water Sanit. Hyg. Dev. 6, 342–347 (2016).
Gattringer, H. et al. Novel vertical ecosystem for sustainable water treatment and reuse in tourist resorts. Int. J. Sustain. Dev. Plan. 11, 263–274 (2016).
Ramprasad, C., Smith, C. S., Memon, F. A. & Philip, L. Removal of chemical and microbial contaminants from greywater using a novel constructed wetland: GROW. Ecol. Eng. 106, 55–65 (2017).
Chowdhury, R., Abaya, J. S., & United Arab Emirates University. An Experimental Study of Greywater Irrigated Green Roof Systems in an Arid Climate. J. Water Manag. Model. https://doi.org/10.14796/JWMM.C437 (2018).
Williams, R. F. et al. Constructed wetlands for urban grey water recycling. Int. J. Environ. Pollut. 33, 93 (2008).
Svete, L. E. Vegetated greywater treatment walls : design modifications for intermittent media filters. 57 (2012).
Dal Ferro, N. et al. Green walls to treat kitchen greywater in urban areas: Performance from a pilot-scale experiment. Sci. Total Environ. 757, 144189 (2021).
Lakho, F. H. et al. Performance of a green wall (Total Value WallTM) at high greywater loading rates and Life Cycle Impact Assessment. Sci. Total Environ. 821, 153470 (2022).
Patil, Y. M. & Munavalli, G. R. Performance evaluation of an Integrated On-site Greywater Treatment System in a tropical region. Ecol. Eng. 95, 492–500 (2016).
Pradhan, S., Helal, M. I., Al-Ghamdi, S. G. & Mackey, H. R. Performance evaluation of various individual and mixed media for greywater treatment in vertical nature-based systems. Chemosphere 245, 125564 (2020).
Chen, H. et al. A critical review on microbial ecology in the novel biological nitrogen removal process: Dynamic balance of complex functional microbes for nitrogen removal. Sci. Total Environ. 857, 159462 (2023).
Prodanovic, V., Zhang, K., Hatt, B., McCarthy, D. & Deletic, A. Optimisation of lightweight green wall media for greywater treatment and reuse. Build. Environ. 131, 99–107 (2018).
Shrouf, A. Hydroponics, Aeroponic and Aquaponic as Compared with Conventional. Farming 27, 247–255 (2017).
Wolcott, S., Martin, P., Goldowitz, J. & Sadeghi, S. Performance of green wall treatment of brewery wastewater. Environ. Prot. Eng. 42, (2016).
McDonald, A. J. S. Nutrient supply and plant growth. In Physiology, Growth and Development of Plants in Culture (eds. Lumsden, P. J., Nicholas, J. R. & Davies, W. J.) 47–57 (Springer Netherlands, 1994). https://doi.org/10.1007/978-94-011-0790-7_4.
Nakhla, G. & Farooq, S. Simultaneous nitrification–denitrification in slow sand filters. J. Hazard. Mater. 96, 291–303 (2003).
Arias, C. A., Del Bubba, M. & Brix, H. Phosphorus removal by sands for use as media in subsurface flow constructed reed beds. Water Res. 35, 1159–1168 (2001).
Verma, S., Daverey, A. & Sharma, A. Slow sand filtration for water and wastewater treatment – a review. Environ. Technol. Rev. 6, 47–58 (2017).
Dalahmeh, S. S. et al. Effects of changing hydraulic and organic loading rates on pollutant reduction in bark, charcoal and sand filters treating greywater. J. Environ. Manag. 132, 338–345 (2014).
Shaikh, I. N. & Ahammed, M. M. Effect of operating mode on the performance of sand filters treating greywater. Environ. Sci. Pollut. Res. 28, 38209–38223 (2021).
Ranjan, P. & Prem, M. Schmutzdecke- A Filtration Layer of Slow Sand Filter. Int. J. Curr. Microbiol. Appl. Sci. 7, 637–645 (2018).
Vuppaladadiyam, A. K. et al. A review on greywater reuse: quality, risks, barriers and global scenarios. Rev. Environ. Sci. Biotechnol. 18, 77–99 (2019).
McQuillan, R. V., Stevens, G. W. & Mumford, K. A. The electrochemical regeneration of granular activated carbons: A review. J. Hazard. Mater. 355, 34–49 (2018).
Albalawneh, A. & Chang, T.-K. Review of the Greywater and proposed Greywater recycling scheme for agricultural irrigation reuses. Int. J. Res. Granthaalayah 3, 16–35 (2015).
Edwin, G. A., Gopalsamy, P. & Muthu, N. Characterization of domestic gray water from point source to determine the potential for urban residential reuse: a short review. Appl. Water Sci. 4, 39–49 (2014).
Yadav, R. K., Sahoo, S. & Patil, S. A. Performance evaluation of the integrated hydroponics-microbial electrochemical technology (iHydroMET) for decentralized domestic wastewater treatment. Chemosphere 288, 132514 (2022).
Yadav, R. K., Siddharth & Patil, S. A. Integrated Hydroponics-Microbial Electrochemical Technology (iHydroMET) is promising for Olericulture along with domestic wastewater management. Bioresour. Technol. Rep. 22, 101428 (2023).
Meshram, A. & Srivastava, N. Epipremnum aureum (Jade Pothos): a multipurpose plant with its medicinal and pharmacological properties. J. Crit. Rev. 2, 5 (2015).
Yadav, R. K., Sahoo, S., Yadav, A. K. & Patil, S. A. Epipremnum aureum is a promising plant candidate for developing nature-based technologies for nutrients removal from wastewaters. J. Environ. Chem. Eng. 9, 106134 (2021).
Awang Chemical and Physical Characteristics of Cocopeat-Based Media Mixtures and Their Effects on the Growth and Development of Celosia cristata. Am. J. Agric. Biol. Sci. 4, 63–71 (2009).
Real, F. J., Benitez, F. J., Acero, J. L. & Casas, F. Adsorption of selected emerging contaminants onto PAC and GAC: Equilibrium isotherms, kinetics, and effect of the water matrix. J. Environ. Sci. Health 52, 727–734 (2017).
S.A.P. acknowledges the financial support from IISER Mohali and the Department of Science and Technology, Government of India (Project No. DST/TMD-EWO/WTI/2K19/EWFH/2019/109). R.K.Y. is grateful to CSIR India for the doctoral scholarship.
Department of Earth and Environmental Sciences, Indian Institute of Science Education and Research Mohali (IISER Mohali), Knowledge City, Sector 81, SAS Nagar, 140306, Punjab, India
Ravi K. Yadav, Siddhant Sahoo & Sunil A. Patil
CSIR-Institute of Minerals and Materials Technology, Bhubaneswar, 751013, India
You can also search for this author in PubMed Google Scholar
You can also search for this author in PubMed Google Scholar
You can also search for this author in PubMed Google Scholar
You can also search for this author in PubMed Google Scholar
R.K.Y.: Design, conceptualization, investigation, software, data analysis, writing—original draft & editing. S.S.: Design, investigation, software, analysis. A.K.Y.: Conceptualization, reviewing, writing—review & editing. S.A.P.: Conceptualization, design, supervision, writing—review & editing, funding acquisition.
Correspondence to Sunil A. Patil.
The authors declare no competing interests but the following competing interests. A design patent titled “A mountable apparatus for treating wastewater at point sources” with Application No. 202211026332 has been filed to the Indian patent office. Applicant: Indian Institute of Science Education and Research Mohali, Inventors: Sunil Anil Patil, Ravi Kumar Yadav, Siddhant Sahoo and Asheesh Kumar Yadav. Status: Pending.
Publisher’s note Springer Nature remains neutral with regard to jurisdictional claims in published maps and institutional affiliations.
Open Access This article is licensed under a Creative Commons Attribution 4.0 International License, which permits use, sharing, adaptation, distribution and reproduction in any medium or format, as long as you give appropriate credit to the original author(s) and the source, provide a link to the Creative Commons license, and indicate if changes were made. The images or other third party material in this article are included in the article’s Creative Commons license, unless indicated otherwise in a credit line to the material. If material is not included in the article’s Creative Commons license and your intended use is not permitted by statutory regulation or exceeds the permitted use, you will need to obtain permission directly from the copyright holder. To view a copy of this license, visit http://creativecommons.org/licenses/by/4.0/.
Yadav, R.K., Sahoo, S., Yadav, A.K. et al. Green wall system coupled with slow sand filtration for efficient greywater management at households. npj Clean Water 6, 73 (2023). https://doi.org/10.1038/s41545-023-00285-3
DOI: https://doi.org/10.1038/s41545-023-00285-3
Anyone you share the following link with will be able to read this content:
Sorry, a shareable link is not currently available for this article.
Provided by the Springer Nature SharedIt content-sharing initiative
npj Clean Water (npj Clean Water) ISSN 2059-7037 (online)
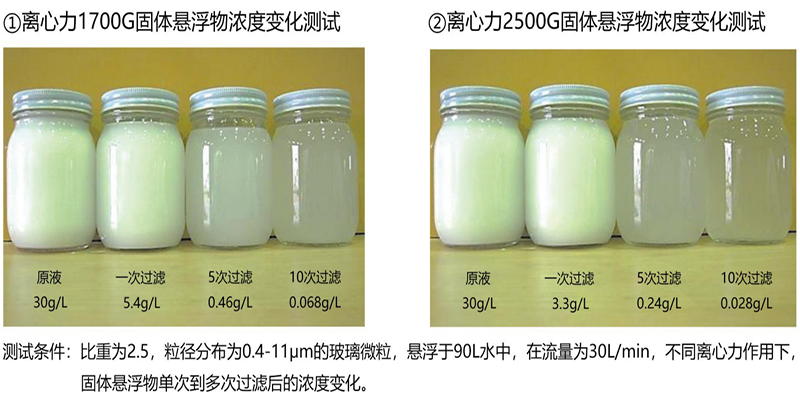
Comb Magnetic Separator Sign up for the Nature Briefing newsletter — what matters in science, free to your inbox daily.